Space & Physics
The various avatars of the Hall effect
In this second article of Ed Publica’s series on the Hall effect, Dr. Saraubh Basu examines the physics of the Hall effect variants discovered over the course of the past century.
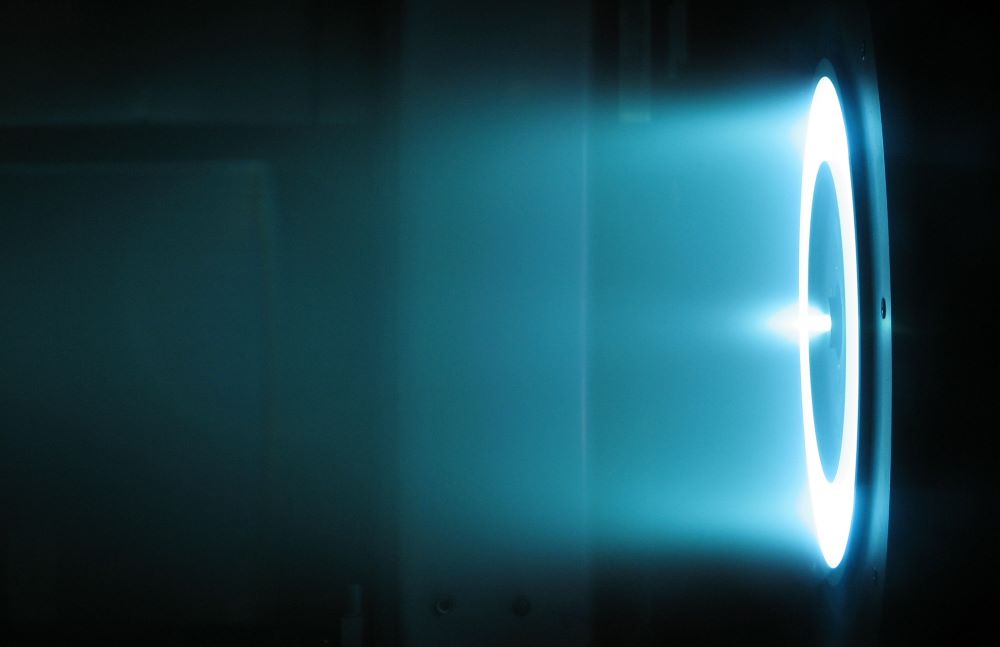
This is the second article of Ed Publica’s series on the Hall effect, which covers the various manifestations of the Hall effect. You can read the first article here.
The ‘anomalous’ Hall effect
In 1881, just two years after Edwin Hall discovered the eponymous Hall effect, he spotted an anomaly when replicating the effect with ferromagnets.
He had observed a tenfold deflection of electric charges this time around, compared to non-magnetic conductors.
Suspecting the magnetic properties played a role, this avatar of the Hall effect is dubbed the anomalous Hall effect. The word ‘anomalous’ is used owing to the fact that external magnetic field no longer remains as a stringent requirement for the Hall effect; instead, the intrinsic magnetization (for instance, the ferromagnet in the above example) fulfils that criterion.

The physicist Edwin Hall. Credit: Wikimedia
The Hall resistivity in ferromagnets increase steeply under the presence of very weak magnetic fields. However, in stronger magnetic fields, the Hall resistivity doesn’t increase further very much. This saturation is rather strange, for it is in contrast to the classical Hall effect where the Hall resistivity maintains its steady growth.
There are several other effects that play a crucial role in determining the anomalous Hall resistivity, thus making it a complicated phenomenon that physicists lack comprehensive understanding about, in comparison to the various other avatars of the Hall effect.
Quantum avatar(s)
The fact that a simple lab experiment showed how the Hall resistivity can be expressed as an equation that contains merely constants, opened up a a plethora of research to understand the cause of this ‘universality’. For it hinted to the involvement of a very fundamental phenomenon.
In 1980, Klaus von Klitzing discovered the quantum avatar of the Hall effect was detected. He was amidst research at a magnetic facility in Grenoble, France, working to improve electron mobility in metal oxide semiconductor field effect transistors (MOSFET). These are transistors that typically operate at extremely low temperatures and under intense magnetic fields.
von Klitzing observed his sample’s Hall resistivity assuming discretized values. This means the resistivity jumps in steps, by a fixed amount that can be scaled as multiples of an integer number (includes 0 along with whole numbers such as 1,2,3, and so on). This discretization reveals the underlying quantum mechanical behavior that has been unraveled at long last – thus bearing its name – the integer quantum Hall effect. von Klitzing later won the Nobel Prize in Physics for 1985 for this work.
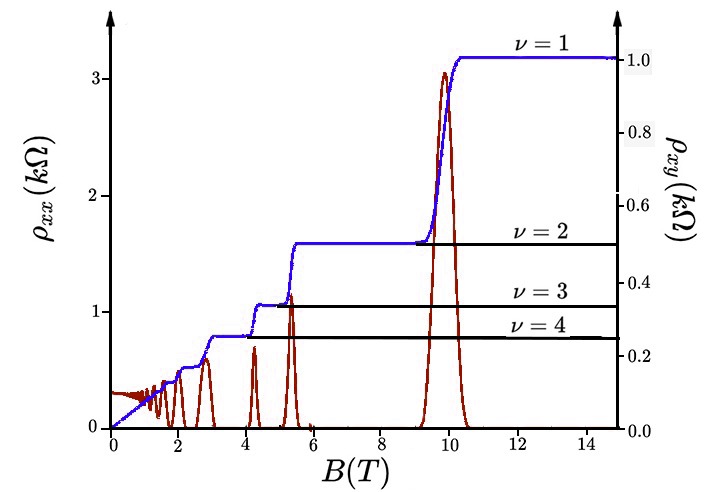
The plot here depicts the transverse and longitudinal Hall resistivity (y-axis) increasing in integer steps as the magnetic field (x-axis) increases. This is due to the integer quantum Hall effect. Credit: Wikimedia
But the quantization isn’t limited to integer multiples. In fact, two years later, the fractional quantum Hall effect was observed in experiments. It was shown there were about 100 fractions, including those that aren’t whole numbers that were now in the formula.
Robert Laughlin, who would later win a share of the 1998 Nobel Prize in Physics, proposed a theory to explain the observations. It boils down to the interaction among electrons, either due to the Coulombic repulsion force or the Pauli exclusion principle.
These interacts would eventually split the degeneracy of these enormously degenerate Landau energy levels. These are quantum states occupied by electrons that complete circular revolutions under the influence of an external magnetic field. Splitting these degeneracies, lead to the opening of an energy gap, for the fractional quantum Hall effect to be observed.
‘Spin’ avatar(s)
Just as there are electric charges in nature, so are there spin currents found in nature. ‘Spin’ is a key property found in quantum particles. Unlike what the name suggests, these quantum particles don’t spin or rotate about any axis passing through them. However, these particles carry an angular momentum as though it does spin.
In 1971, before von Klitzing observed the quantum Hall effect, Mikhail Dyakonov and Vladimir Perel hypothesized the spin Hall effect.
In this avatar of the Hall effect, quantum spins of opposite kinds accumulate at the edges of the sample, orthogonal to the direction in which the charge current passes.
The spin selection can be facilitated by the spin-orbit coupling. This refers to the modified energy levels in an atom when the electron’s motion is under the influence on the magnetic field generated by the nucleus. Strong coupling may be intrinsic to doped semiconductors. The proposal has triggered intense investigation of the phenomenon, with first experimental observations of the spin Hall effect seen in n-doped semiconductors and two-dimensional hole gases.
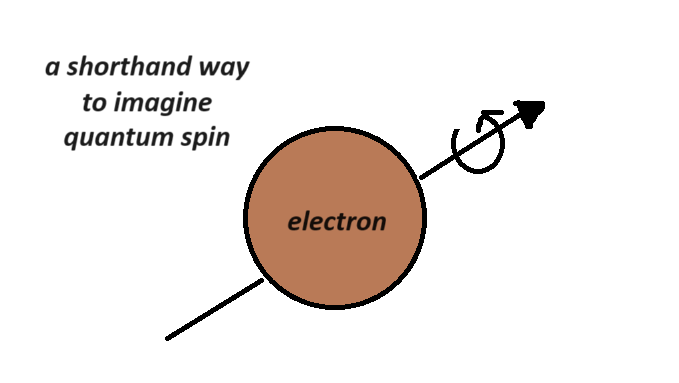
Quantum spins don’t really look like the depiction above, which is meant to showcase a fact that particles like electrons do have an intrinsic angular momentum nonetheless. Credit: Karthik / Ed Publica
For more than a decade, studies concerning the spin current and its application to novel spintronics (or spin electronics) have received plethora of attention. This is with regard to efficiently generating, manipulating and detecting spin accumulation in a sample material. Some progress has also occurred from the device fabrication perspective via techniques such as spin injection, among others.
A major advantage in dealing with the spin current lies in the non-dissipative (or very less dissipation) nature which arises owing to the time reversal invariance of the spin current. This presents a non-dissipative scenario (unlike the dissipative effects seen with charged currents), thus making it quite advantageous for spin transport phenomena.
Furthermore, a quantized version of the spin Hall effect exists, with mercury telluride and cadmium telluride quantum well superlattices, showcasing this effect. In 2005, a quantum treatment was proposed by Charles Kane and Eugene Mele, in the form of a tight binding toy model of electrons operating in a two-dimensional honeycomb lattice.
In fact, the ‘wonder material’ graphene, which is a two-dimensional honeycomb lattice constituting carbon atoms, does satisfy some key requirements for the quantum spin Hall effect. However, it lacks a large spin-orbit coupling among other requirements.
Nonetheless, graphene’s ability to entertain the quantum spin Hall effect, makes it a prospective candidate to find applications in next-generation spintronic devices.
Space & Physics
MIT unveils an ultra-efficient 5G receiver that may supercharge future smart devices
A key innovation lies in the chip’s clever use of a phenomenon called the Miller effect, which allows small capacitors to perform like larger ones
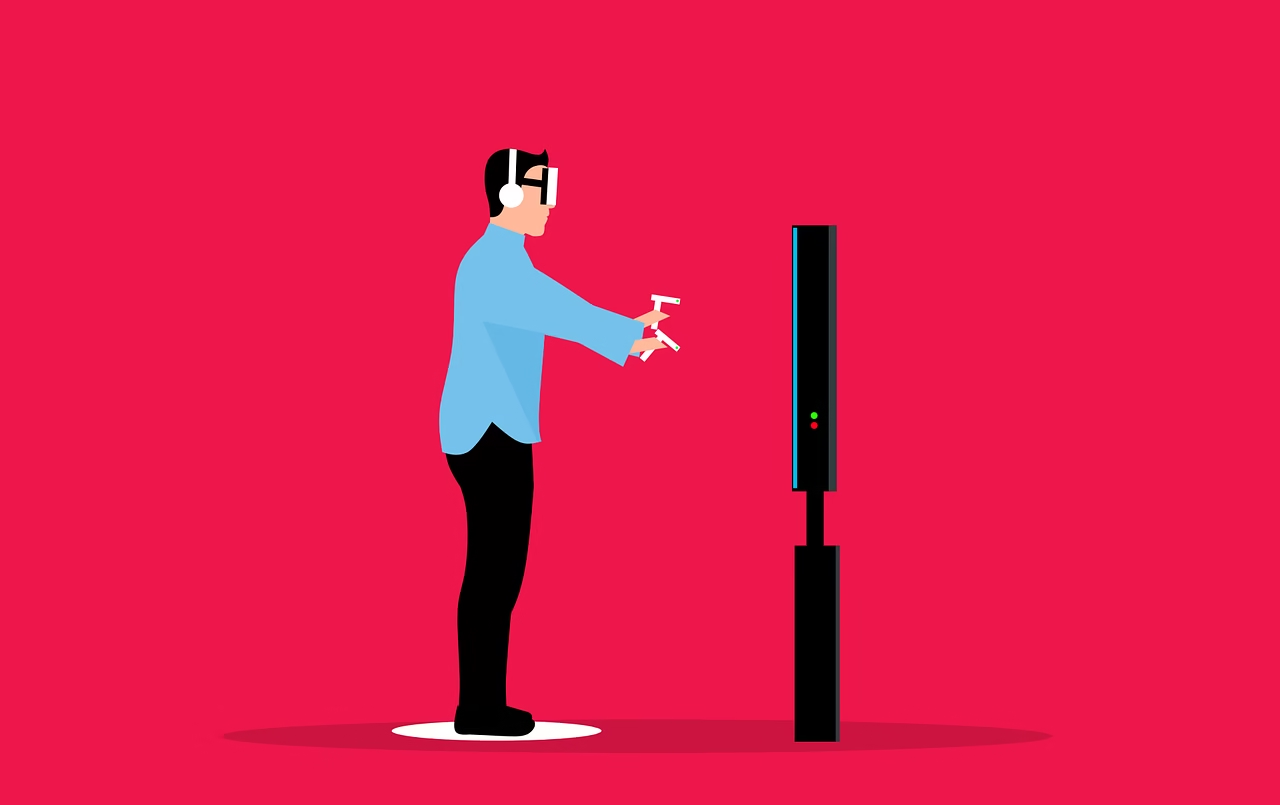
A team of MIT researchers has developed a groundbreaking wireless receiver that could transform the future of Internet of Things (IoT) devices by dramatically improving energy efficiency and resilience to signal interference.
Designed for use in compact, battery-powered smart gadgets—like health monitors, environmental sensors, and industrial trackers—the new chip consumes less than a milliwatt of power and is roughly 30 times more resistant to certain types of interference than conventional receivers.
“This receiver could help expand the capabilities of IoT gadgets,” said Soroush Araei, an electrical engineering graduate student at MIT and lead author of the study, in a media statement. “Devices could become smaller, last longer on a battery, and work more reliably in crowded wireless environments like factory floors or smart cities.”
The chip, recently unveiled at the IEEE Radio Frequency Integrated Circuits Symposium, stands out for its novel use of passive filtering and ultra-small capacitors controlled by tiny switches. These switches require far less power than those typically found in existing IoT receivers.
A key innovation lies in the chip’s clever use of a phenomenon called the Miller effect, which allows small capacitors to perform like larger ones. This means the receiver achieves necessary filtering without relying on bulky components, keeping the circuit size under 0.05 square millimeters.
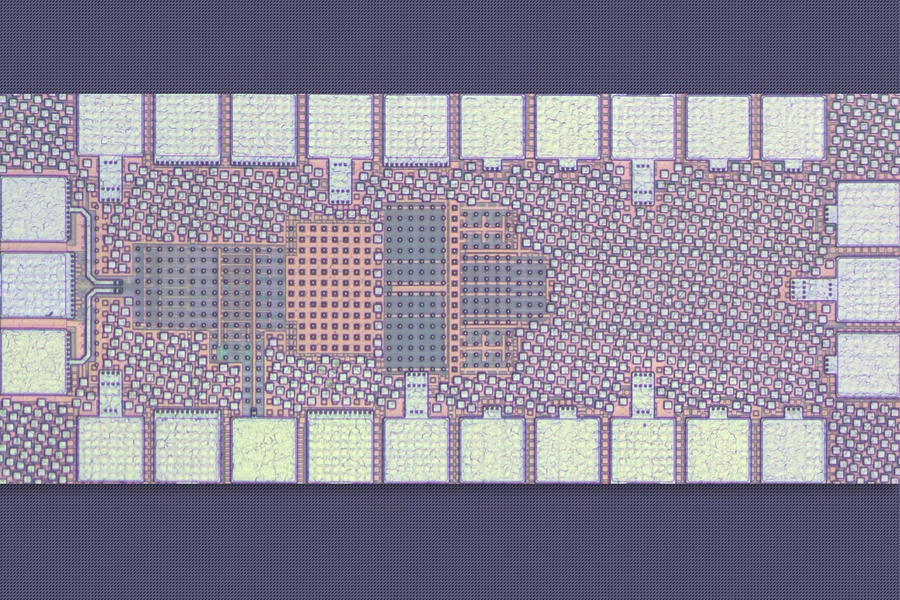
Traditional IoT receivers rely on fixed-frequency filters to block interference, but next-generation 5G-compatible devices need to operate across wider frequency ranges. The MIT design meets this demand using an innovative on-chip switch-capacitor network that blocks unwanted harmonic interference early in the signal chain—before it gets amplified and digitized.
Another critical breakthrough is a technique called bootstrap clocking, which ensures the miniature switches operate correctly even at a low power supply of just 0.6 volts. This helps maintain reliability without adding complex circuitry or draining battery life.
The chip’s minimalist design—using fewer and smaller components—also reduces signal leakage and manufacturing costs, making it well-suited for mass production.
Looking ahead, the MIT team is exploring ways to run the receiver without any dedicated power source—possibly by harvesting ambient energy from nearby Wi-Fi or Bluetooth signals.
The research was conducted by Araei alongside Mohammad Barzgari, Haibo Yang, and senior author Professor Negar Reiskarimian of MIT’s Microsystems Technology Laboratories.
Society
Ahmedabad Plane Crash: The Science Behind Aircraft Take-Off -Understanding the Physics of Flight
Take-off is one of the most critical phases of flight, relying on the precise orchestration of aerodynamics, propulsion, and control systems. Here’s how it works:
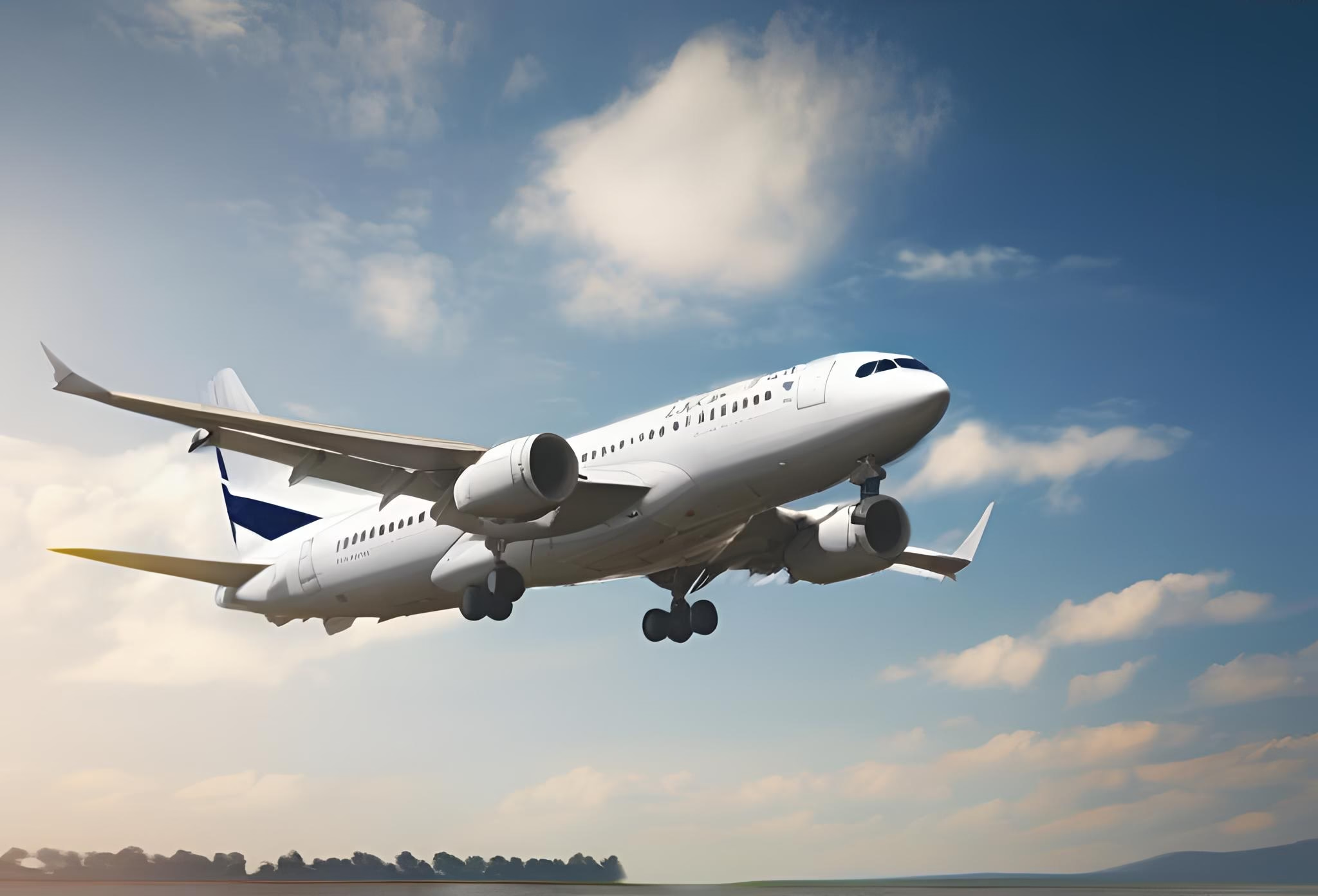
On June 12, 2025, a tragic aviation accident struck Ahmedabad, India when a regional passenger aircraft, Air India flight A1-171, crashed during take-off at Sardar Vallabhbhai Patel International Airport. According to preliminary reports, the incident resulted in over 200 confirmed casualties, including both passengers and crew members, and several others are critically injured. The aviation community and scientific world now turn their eyes not just toward the cause but also toward understanding the complex science behind what should have been a routine take-off.
How Do Aircraft Take Off?
Take-off is one of the most critical phases of flight, relying on the precise orchestration of aerodynamics, propulsion, and control systems. Here’s how it works:
1. Lift and Thrust
To leave the ground, an aircraft must generate lift, a force that counters gravity. This is achieved through the unique shape of the wing, called an airfoil, which creates a pressure difference — higher pressure under the wing and lower pressure above — according to Bernoulli’s Principle and Newton’s Third Law.
Simultaneously, engines provide thrust, propelling the aircraft forward. Most commercial jets use turbofan engines, which accelerate air through turbines to generate power.
2. Critical Speeds
Before takeoff, pilots calculate critical speeds:
- V1 (Decision Speed): The last moment a takeoff can be safely aborted.
- Vr (Rotation Speed): The speed at which the pilot begins to lift the nose.
- V2 (Takeoff Safety Speed): The speed needed to climb safely even if one engine fails.
If anything disrupts this process — like bird strikes, engine failure, or runway obstructions — the results can be catastrophic.
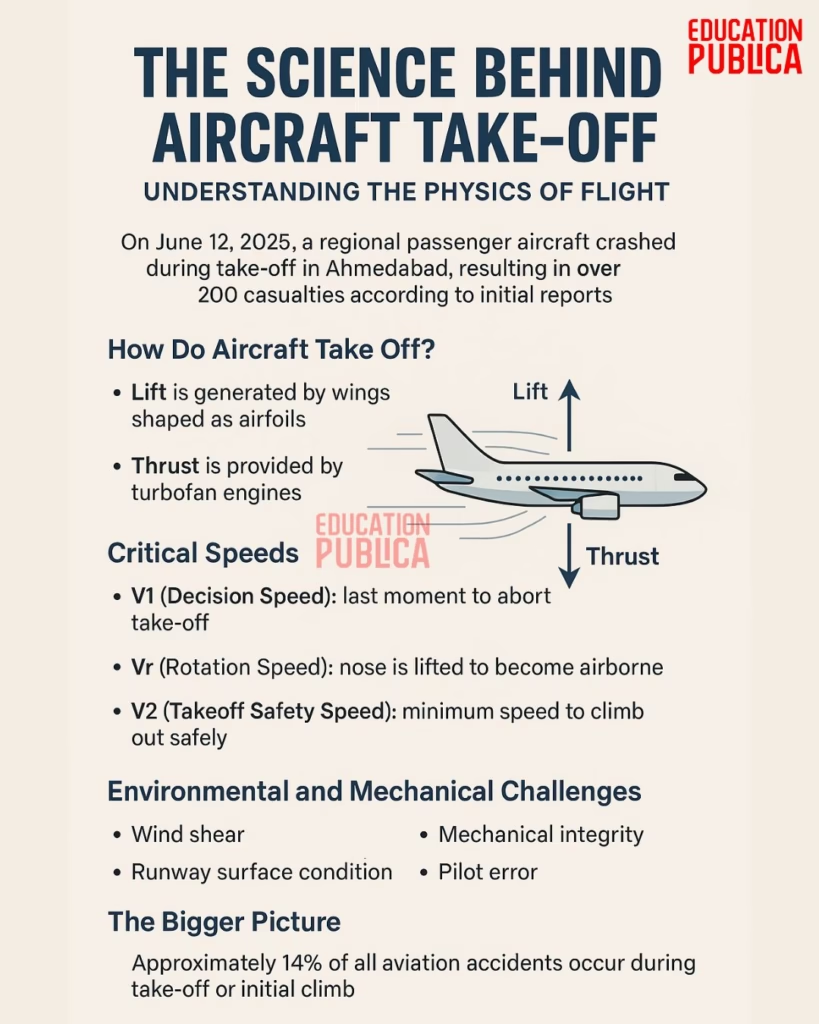
Environmental and Mechanical Challenges
Factors like wind shear, runway surface condition, mechanical integrity, or pilot error can interfere with safe take-off. Investigators will be analyzing these very aspects in the Ahmedabad case.
The Bigger Picture
Take-off accounts for a small fraction of total flight time but is disproportionately associated with accidents — approximately 14% of all aviation accidents occur during take-off or initial climb.
Space & Physics
MIT claims breakthrough in simulating physics of squishy, elastic materials
In a series of experiments, the new solver demonstrated its ability to simulate a diverse array of elastic behaviors, ranging from bouncing geometric shapes to soft, squishy characters
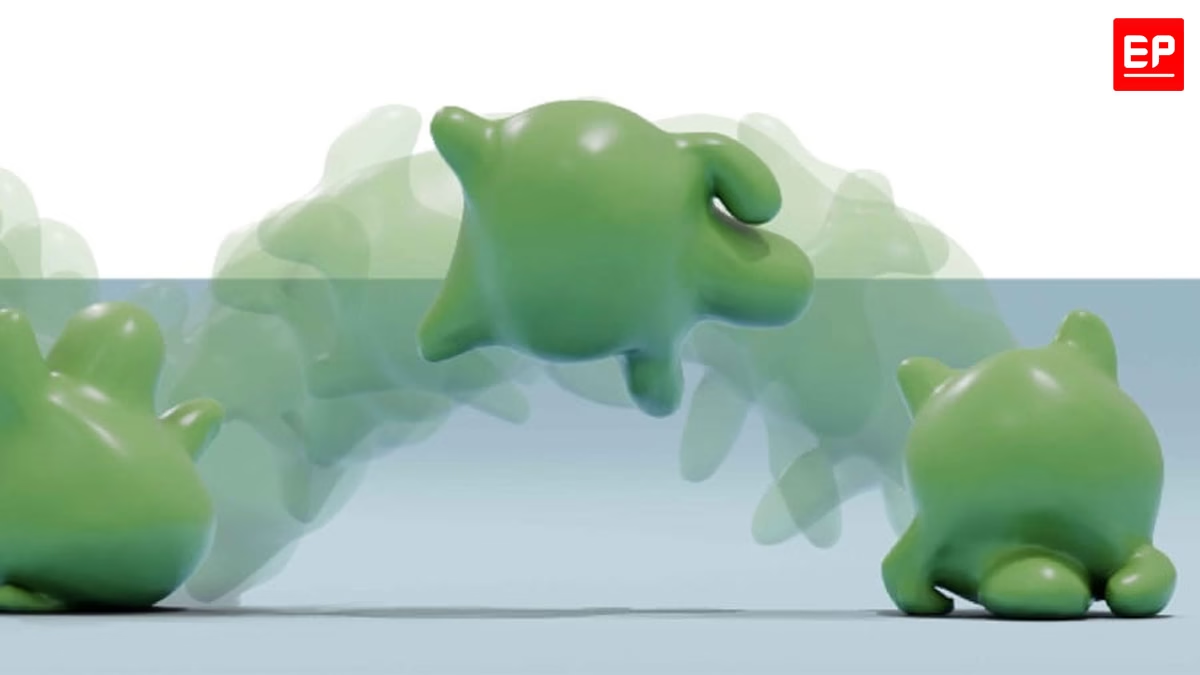
Researchers at MIT claim to have unveiled a novel physics-based simulation method that significantly improves stability and accuracy when modeling elastic materials — a key development for industries spanning animation, engineering, and digital fabrication.
In a series of experiments, the new solver demonstrated its ability to simulate a diverse array of elastic behaviors, ranging from bouncing geometric shapes to soft, squishy characters. Crucially, it maintained important physical properties and remained stable over long periods of time — an area where many existing methods falter.
Other simulation techniques frequently struggled in tests: some became unstable and caused erratic behavior, while others introduced excessive damping that distorted the motion. In contrast, the new method preserved elasticity without compromising reliability.
“Because our method demonstrates more stability, it can give animators more reliability and confidence when simulating anything elastic, whether it’s something from the real world or even something completely imaginary,” Leticia Mattos Da Silva, a graduate student at MIT’s Department of Electrical Engineering and Computer Science, said in a media statement.
Their study, though not yet peer-reviewed or published, will be presented at the August proceedings of the SIGGRAPH conference in Vancouver, Canada.
While the solver does not prioritize speed as aggressively as some tools, it avoids the accuracy and robustness trade-offs often associated with faster methods. It also sidesteps the complexity of nonlinear solvers, which are commonly used in physics-based approaches but are often sensitive and prone to failure.
Looking ahead, the research team aims to reduce computational costs and broaden the solver’s applications. One promising direction is in engineering and fabrication, where accurate elastic simulations could enhance the design of real-world products such as garments, medical devices, and toys.
“We were able to revive an old class of integrators in our work. My guess is there are other examples where researchers can revisit a problem to find a hidden convexity structure that could offer a lot of advantages,” Mattos Da Silva added.
The study opens new possibilities not only for digital content creation but also for practical design fields that rely on predictive simulations of flexible materials.
-
Society4 months ago
Starliner crew challenge rhetoric, says they were never “stranded”
-
Space & Physics3 months ago
Could dark energy be a trick played by time?
-
Earth4 months ago
How IIT Kanpur is Paving the Way for a Solar-Powered Future in India’s Energy Transition
-
Space & Physics3 months ago
Sunita Williams aged less in space due to time dilation
-
Learning & Teaching4 months ago
Canine Cognitive Abilities: Memory, Intelligence, and Human Interaction
-
Earth2 months ago
122 Forests, 3.2 Million Trees: How One Man Built the World’s Largest Miyawaki Forest
-
Women In Science3 months ago
Neena Gupta: Shaping the Future of Algebraic Geometry
-
Society5 months ago
Sustainable Farming: The Microgreens Model from Kerala, South India